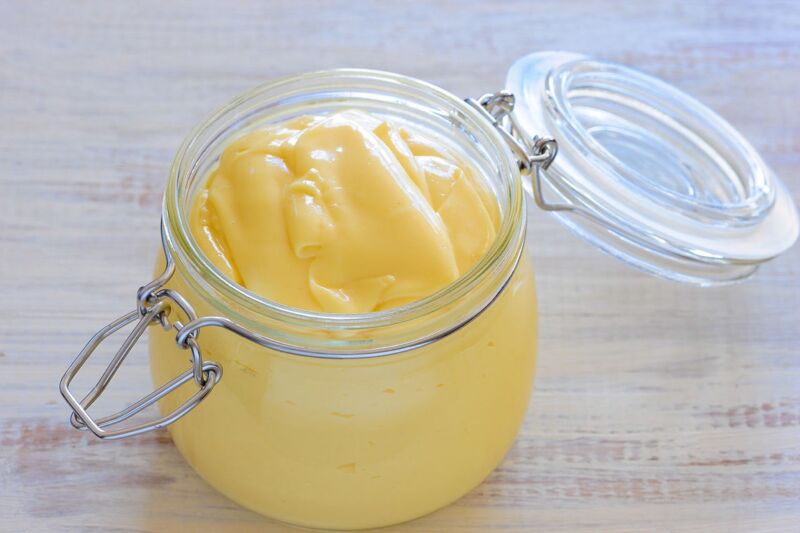
Inertial confinement fusion is a method of generating energy through nuclear fusion that has been plagued by a number of scientific challenges (although progress is being made). Researchers at LeHigh University are attempting to overcome one particular irritation with this approach by conducting experiments using mayonnaise in a rotating figure-eight device. They describe their latest findings in a new paper published in the journal Physical Review E with an eye toward increasing the energy yields of fusion.
The work builds on previous research in the LeHigh lab of mechanical engineer Arindam Banerjee, who focuses on investigating the dynamics of fluids and other materials in response to extremely high acceleration and centrifugal force. In this case, his team investigated what’s known as the “instability threshold” of elastic/plastic materials. Scientists have debated whether this is due to initial conditions or the result of “more local catastrophic processes,” Banerjee said. The question is relevant to several fields, including geophysics, astrophysics, explosive welding and, yes, inertial confinement fusion.
How exactly does inertial confinement fusion work? As Chris Lee explained for Ars in 2016:
The idea behind inertial confinement fusion is simple. To fuse two atoms, you need to bring their nuclei into contact with each other. Both nuclei are positively charged, so they repel each other, meaning that force is needed to convince two hydrogen nuclei to touch. In a hydrogen bomb, force is generated when a small fission bomb explodes, compressing a nucleus of hydrogen. This fuses to create heavier elements, releasing a huge amount of energy.
Because they’re game changers, scientists prefer not to detonate nuclear weapons every time they want to study nuclear fusion or use it to generate electricity. Which brings us to inertial confinement fusion. In inertial confinement fusion, the hydrogen nucleus consists of a spherical ball of hydrogen ice in a heavy metal shell. The shell is illuminated by powerful lasers, which burn off much of the material. The reaction force of the vaporized material exploding outward causes the remaining shell to implode. The resulting shock wave compresses the center of the hydrogen nucleus, causing it to fuse.
If the confinement fusion were to end there, the amount of energy released would be small. But the energy released by the initial fusion burn in the center generates enough heat for the hydrogen on the outside of the pellet to reach the required temperature and pressure. So eventually (at least in computer models) all the hydrogen is consumed in a fiery death, and enormous amounts of energy are released.
That's the idea anyway. The problem is that hydrodynamic instabilities tend to form in the plasma state – Banerjee likens it to “two materials [that] each other like “fingers” in the presence of gravity or an accelerating field – which in turn reduces the energy yields. The technical term is a Rayleigh-Taylor instability, which occurs between two materials of different densities, where the density and pressure gradients move in opposite directions. Mayonnaise turns out to be an excellent analogue for investigating this instability in accelerated solids, without the need for a laboratory setup with high temperature and pressure conditions, because it is a non-Newtonian fluid.
“We use mayonnaise because it behaves like a solid, but when it's exposed to a pressure gradient, it starts to flow,” Banerjee said. “Just like a traditional molten metal, if you put stress on mayonnaise, it will deform, but when you remove the stress, it goes back to its original shape. So there's an elastic phase followed by a stable plastic phase. The next phase is when it starts to flow, and that's where the instability starts.”
More mayonnaise, please
2019 video showing the Rayleigh Taylor instability experiment with a rotating wheel at Lehigh University.
His team’s 2019 experiments involved pouring Hellman’s Real Mayonnaise (no Miracle Whip for this crew) into a Plexiglas container and then creating wave-like disturbances in the mayo. One experiment involved placing the container on a rotating figure-eight wheel and tracking the material with a high-speed camera, using an image-processing algorithm to analyze the images. Their results supported the claim that the instability threshold depends on the initial conditions, namely amplitude and wavelength.
This final paper sheds more light on the structural integrity of fusion capsules used in inertial confinement fusion, looking more closely at the material properties, amplitude and wavelength conditions, and the acceleration rate of such materials when they reach the Rayleigh-Taylor instability threshold. The more scientists know about the phase transition from the elastic to the stable phase, the better they can control the conditions and maintain an elastic or plastic phase, thus avoiding the instability. Banerjee et al. were able to identify the conditions to maintain the elastic phase, which may be important for the design of future pellets for inertial confinement fusion.
That said, the mayonnaise experiments are an analogue, orders of magnitude removed from the real conditions of nuclear fusion, as Banerjee readily acknowledges. He is nonetheless hopeful that future research will improve the predictability of exactly what happens inside the pellets in their high-temperature, high-pressure environments. “We are another cog in this giant wheel of researchers,” he said. “And we are all working to make inertial fusion cheaper and therefore feasible.”
DOI: Physical Review E, 2024. 10.1103/PhysRevE.109.055103 (About DOIs).